Searching for a needle in a needle-stack
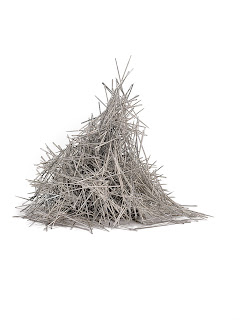
Whole-genome sequencing is a game-changer for human genetics. It is now possible to deduce every base of an individual’s genome (all 6 billion of them – two copies of 3 billion each) for a couple of thousand euros, and dropping. (Yes, euros). Even Ozzy Osbourne just got his genome sequenced! For researchers searching for the causes of genetic disease (or resistance to vast quantities of drugs and alcohol), this means they no longer have to infer where a mutation is by tracking a sampling of “markers” spaced across the genome – they can directly see all of the genetic information. The problem is, they directly see all of the genetic information. If each of us carries thousands of mutations – changes that are very rare or may even have never been seen before in any other person – then telling which one of those changes is actually causing the condition is a tough task. Researchers in psychiatric genetics are currently grappling with how to handle this glut of information. The pr