Genetic entropy and the human intellect (or why we're not getting dumber)
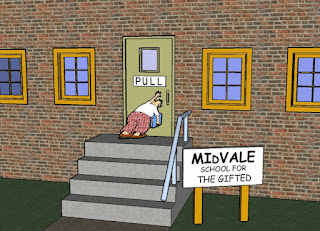
This is a modified version of a letter published in Trends in Genetics: Kevin J. Mitchell (2012). Genetic entropy and the human intellect. Trends in Genetics, 14 th December 2012. Two articles by Gerald Crabtree float the notion that we, as a species, are gradually declining in average intellect, due to the accumulation of mutations that deleteriously affect brain development or function [ 1 , 2 ] . The observations that prompted this view seem to be: (i) intellectual disability can be caused by mutations in any one of a very large number of genes, and: (ii) de novo mutations arise at a low but steady rate in every new egg or sperm. He further proposes that: (iii) genes involved in brain development or function are especially vulnerable to the effects of such mutations. Considered in isolation, these could reasonably lead to the conclusion that mutations reducing intelligence must be constantly accumulating in the human gene pool. Thankfully, these f