Wired for Sex
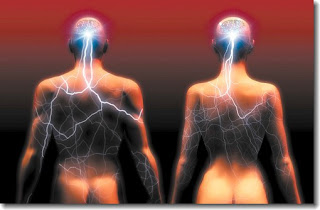
Male and female brains are wired differently. That is not intended metaphorically – they literally have different amounts and/or patterns of axonal connections between a variety of brain regions, as well as differences in the size or number of cells in various regions. This is true in mammals, birds, fish, even insects and correlates with hard-wired, innate differences in behaviour between the sexes across in species across all these phyla. This is as true for humans as for any other species. The behaviours that show the most robust and innate differences between the sexes are involved in mating, reproduction, parental behaviour, territoriality and aggression and it is the brain areas that control these behaviours that are the most obviously sexually dimorphic (showing a difference in size or morphology between the sexes). In mammals, these include areas in the limbic system, including parts of the hypothalamus, amygdala, preoptic area and bed nucleus of the stria terminali...